ITER is a Latin word meaning “the way.” It’s also the name of a mammoth energy project sprawled over 180 hectares (440acres) in the South of France. It’s the International Thermonuclear Experimental Reactor project. It aims to debug and prove the technology that will bring nuclear fusion, the same process that powers the sun and stars, to mankind. If successful, it will pave the way for the design of power plants that are carbon-free and produce no long-lived radioactive waste. Such thermonuclear power plants would give the world nearly inexhaustible energy derived from fusing atoms together, just as the sun has been doing for more than four billion years.
A collaborative effort that began in 1985, ITER employs thousands of scientists and engineers from China, the European Union, India, Japan, Korea, Russia and the United States. Their goal on this 35 year project is to develop and model nuclear fusion technology—to work out the kinks and pave the way for commercial fusion-powered reactors. ITER hopes to “go live” in 2025 and expects it will be able to generate 500 MW of energy. That’s enough to power 175,000 homes. It will provide a “proof of concept” that can guide engineering and construction companies to begin worldwide production of fusion-powered reactors.
Building a Miniature Sun Here on Earth
Scientists understand the physics of our sun and how it can radiate energy for billions of years. Two factors—the combination of colossal gravitational pressure within the sun’s core that squeezes atoms together into a kind of “particle soup,” and a temperature of some fifteen million degrees that accelerates the motion of atoms to phenomenal speeds—these combine to smash atoms so tightly together that they fuse. Four hydrogen atoms are fused to make one Helium atom. In the process, that thermonuclear reaction releases gargantuan amounts of energy that light our skies and warm the earth.
Scientists have long pondered how they could create conditions on earth that would support nuclear fusion. Hydrogen bombs are fusion devices, but they’re hardly suited to peaceful energy production. In 1956 Russian scientists working at the Kurchatov Institute in Moscow developed the concept of a tokamak. The heart of a tokamak is its toroidal (doughnut-shaped) vacuum chamber. Hydrogen is pumped into the chamber and converted from its gaseous state to a very hot plasma. Because there’s no technology that can replicate the intense gravitational field at the core of the sun, scientists have to rely on raising the temperature of the plasma to incredible levels, up to 150 million degrees Centigrade, so fusion can take place.
But there’s a problem with plasma. It’s often called the “fourth state of matter” following solids, liquids and gases. Hydrogen plasma is composed of charged particles that conduct electricity and, at 150 million degrees, melt anything they touch. To prevent catastrophic failure of the tokamak, the plasma has to be contained by something “non-physical.” What science fiction writers would call a “force field.” While it’s extremely challenging to create such fields that will confine 840 cubic meters of intensely hot plasma to a doughnut shape, magnetic fields can do the job.
Enter Super Conductivity
There’s nothing ordinary about a tokamak. To create magnetic fields strong enough to hold the plasma in its doughnut shape, a tokamak uses gigantic electromagnets. They take the form of huge D-shaped coils of wire through which thousands of Amperes of current flow. The tokamak uses 26 mammoth coils, each weighing 360 tonnes; each similar to the mass of a fully loaded Boeing 747-300 aircraft. Each coil is 14 meters high and 9 meters wide. Altogether, the wire in the electromagnet coils is more than 100,000 km (62,000 miles) long. That’s more than enough to circle the earth twice at its equator.
While the plasma “burns” at temperatures ten times hotter than the core of the sun, cryogenics—the science of extremely cold temperatures, close to absolute zero—enters the picture. Certain metals become super conductors when current is applied to them if the temperature is low enough. A superconductor exhibits zero electrical resistance when cooled below a temperature known as its transition temperature. When superconducting wires are cooled below that point, massive electrical currents can be passed through them which, in turn, produce massive magnetic fields not obtainable using ordinary copper conductors. The ITER tokamak uses an alloy of Niobium and Tin (Nb3Sn).
There are more than 500 companies in the U.S. that provide technology and materials to ITER. Based in Lisbon, NH, New England Wire Technologies (NEWT) has built a reputation in the superconductor community for its ability to design and manufacture complex cable constructions for superconductor applications. Their work began in the 60’s when NEWT began manufacturing keystone (trapezoidal) shaped cable for particle accelerator projects. NEWT went on to supply the U.S. Department of Energy’s Princeton Plasma Physics Laboratory with specialized wire and cabling designs used in their thermonuclear fusion research.
The ITER procurement team asked several companies to build a proof of concept prototype cable assembly. The Nb3Sn alloy is extremely brittle and cannot be easily drawn into a wire, which is necessary for winding superconducting electromagnets. In addition, the unit length is long and the cost of the raw material is very high. The final cable has 1422 strands with 900 being superconductor and 522 being copper. Only NEWT was able to successfully manufacture the prototype, which led to the production level contract award from ITER.
Given New England Wire’s experience working with superconductor projects, Director of Engineering, Michael Boivin notes that “We understood the sensitive nature of the material and the care needed to work with it. In addition, the construction required excellent mapping of each strand to ensure not only the correct ratio, but also the positioning of superconductors and copper strands. Each wire looks the same, having a chrome plated surface. Furthermore, the superconductor strand is encased in copper, so even under the plating it looks just like copper. The lay lengths are long, which makes the early phases of the cabling operation challenging. There is also a specific compaction that is necessary with tight tolerances on the overall diameter needed to ensure the cable can be inserted in a stainless steel tube that will be flooded with liquid helium to provide the cryogenic temperatures required for super conductivity.”
The goal of the International Thermonuclear Experimental Reactor project is to generate significantly more energy than it takes to heat plasma to 150 million degrees Centigrade and to supply current to the electromagnets so the thermonuclear reaction can be sustained continuously. ITER has been designed to produce 500 MW of output power for 50 MW of input power—or ten times the amount of energy put in. The current record for net released fusion power is 16 MW, held by the European JET facility located in Culham, UK.
A New World of Energy?
The ITER tokamak will use a small fraction of the heat generated by the 150 million degree plasma to heat water, generate steam and power turbines. That’s what coal-powered, natural gas-powered and nuclear fission plants do today. Harnessing the energy of the sun isn’t easy, but once ITER has laid the groundwork, commercial thermonuclear fusion plants will follow. Learn more about the fascinating ITER project and feel free to contact our consulting engineers at NEWT to discuss your next project, no matter how complex and challenging it might be.
Looking for an update on the ITER project? Read this.
Custom Design
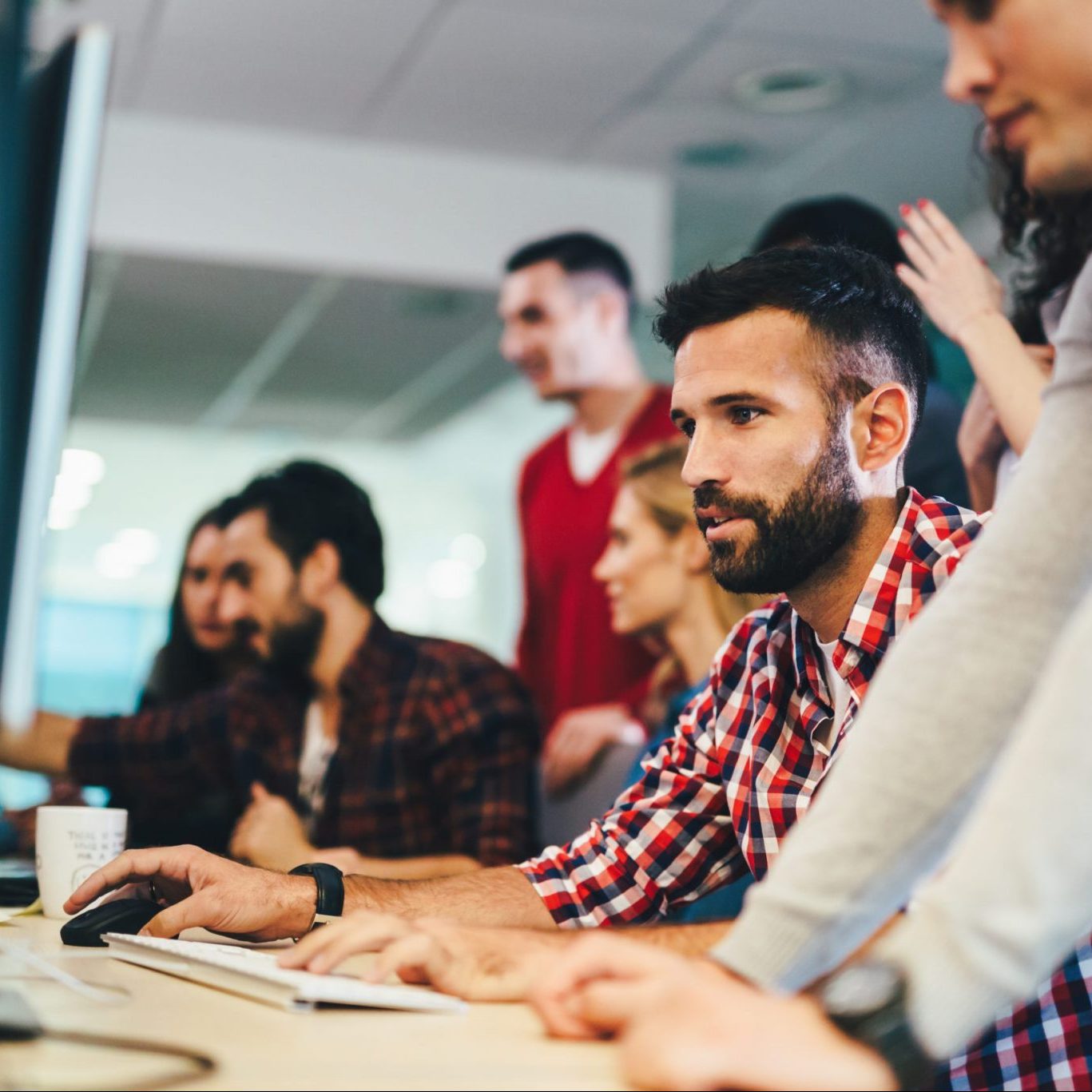
About Our Engineers
Innovative solutions start with an experienced team of engineers, armed with the latest design tools and technology. Our focus on understanding the individual, complex challenges of our customers and designing solutions that fit, is why we have been trusted as the industry leader for over 100 years.